Present | 2040–2060 | 2060–2080 | |
Return period (yr) | 1-in-100 | 1-in-54 | 1-in-43 |
100-year | 7.4 in (189 mm) | 8.6 in (217 mm) | 9.2 in (233 mm) |
Climate Risk Assessment: Barnstable, Massachusetts
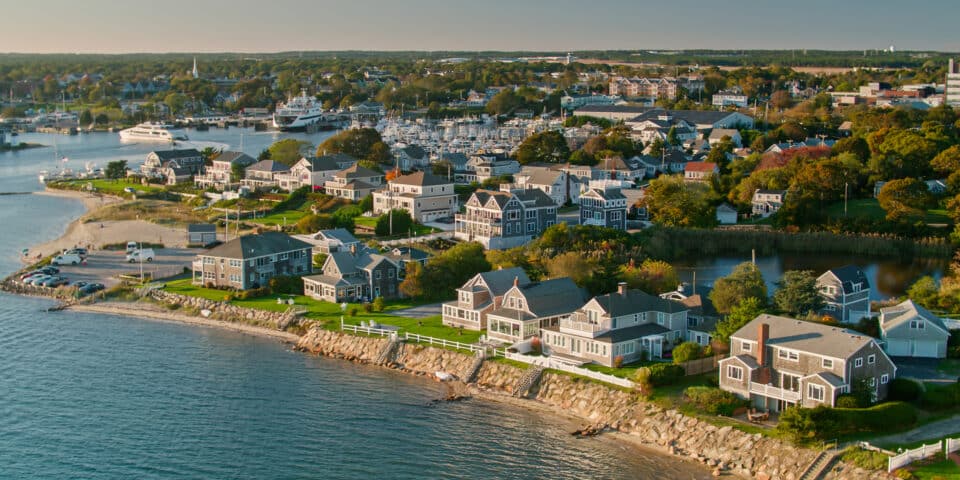
The impacts of climate change on the frequency and severity of physical hazards are putting many communities at risk. As the threat of climate change grows, so too does the need for accessible information, tools, and expertise to support climate-resilient decision making across multiple scales, from communities to countries. Woodwell Climate Research Center believes there is a need to localize and customize climate risk assessments. This information is critical for local government leaders as they make planning decisions, but it is not available to all communities. Woodwell believes that this science should be freely and widely available. To address this gap, Woodwell works with communities across the world, including Barnstable, MA, to provide community climate risk assessments, free of charge.
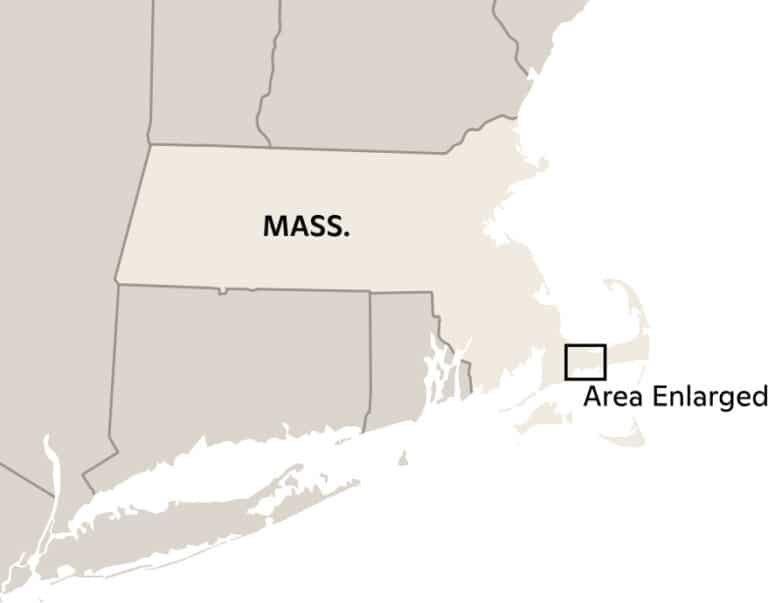
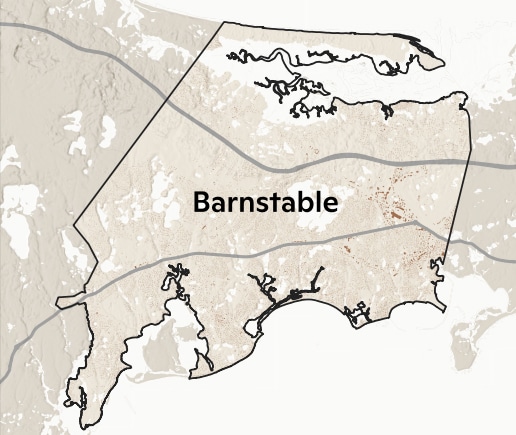
Results summary
As a result of climate change, flood risk is projected to increase for Barnstable. The probability of the historical 100-year rainfall event, a useful indicator of flood risk, is expected to almost double by midcentury and be more than two times more likely by the end of the century. Sea levels are also projected to rise throughout this century with an increase of 1.31 feet (0.4 meters) by 2050 and 2.07 feet (0.63 meters) by 2070. Both sea level rise and heavier rainfall will translate into greater flood depths and extent for Barnstable. The vulnerability of Barnstable’s stormwater system and pump stations were evaluated under the present and future 100-year rainfall event. Low lying roads in Barnstable were also examined to determine areas that are at risk. Lastly, future storm surge risk related to tropical cyclones was evaluated. Here we present our findings on extreme precipitation, flooding, and tropical cyclones storm surge risk to help Barnstable in its plans to create a more resilient future for all residents.
Extreme rainfall
The Fifth National Climate Assessment shows that the U.S. Northeast region has already seen a 60% increase, the largest in the U.S., in annual precipitation occurring from the heaviest 1% of events.1 Future warming is expected to continue this trend of intensification, meaning more frequent and severe rainfall events. Here we use localized future precipitation data from downscaled global climate models to calculate the change in probability of extreme rainfall events. A detailed explanation of the precipitation data processing can be found in the methodology section of this document. In Table 1, we show the changes in the return period of the present-day (2000–2020) 100-year rainfall event for midcentury (2040–2060) and late century (2060–2080). By midcentury, the present-day 100-year event will occur with a return period of 1-in-54. By late century, the present-day 100-year event will become a 1-in-43 year event.
According to the National Atlas 14 published by the National Oceanic and Atmospheric Administration (NOAA), the 100-year rainfall amount, based on present-day rainfall records, for Barnstable is 7.4 inches (189 mm).2 For reference, the present-day annual average rainfall for Barnstable, MA is 47.7 inches (1211 mm).3 By midcentury, the 100-year amount will increase to 8.6 inches (217 mm) and by late century this will further rise to 9.2 inches (233 mm; Table 1).
Table 1. Mid- and late-21st century change in historical 100-year return period and rainfall. The mean future return period in years and rainfall amounts in inches and millimeters for Barnstable of the present-day, 2040–2060, and 2060–2080 100-year rainfall events.
Flooding
For a detailed explanation of the flood model input data and flood modeling procedures, please refer to the methodology section of this document.
Flood extent comparison
Before estimating future flood risk, we compare the present-day flood risk results against the Federal Emergency Management Agency (FEMA) flood maps as a validation exercise. FEMA maps are not ground truth data, but it is useful to compare various model results given the lack of appropriate reference data. Figure 1 shows the differences and similarities between FEMA’s estimate and Woodwell’s estimate of the 100-year flood extent for the Barnstable, MA region. Areas where only FEMA predicts flood risk are shown in green, areas where only Woodwell predicts flood risk are shown in red, and areas where both predict flood risk are shown in purple. Several patterns emerge when comparing the extents visually. The coastal risk estimated by FEMA is greater than Woodwell estimates in some locations, particularly in the southeast at Dunbar Point. This is likely a result of the digital elevation model (DEM) differing from FEMA, as well as the coastal defenses data Barnstable provided to us not being included in the FEMA results. The FEMA report was revised in 2021; however, the coastal flooding in Barnstable County was analyzed in 2013, so any changes in the last decade also wouldn’t be reflected.4 Finally, FEMA shows no flood risk in areas disconnected from coastal regions, also known as pluvial flooding, while Woodwell demonstrates extensive pluvial areas are vulnerable to flooding such as southwest of the Cape Cod Gateway Airport. This is because FEMA does not account for pluvial flooding.
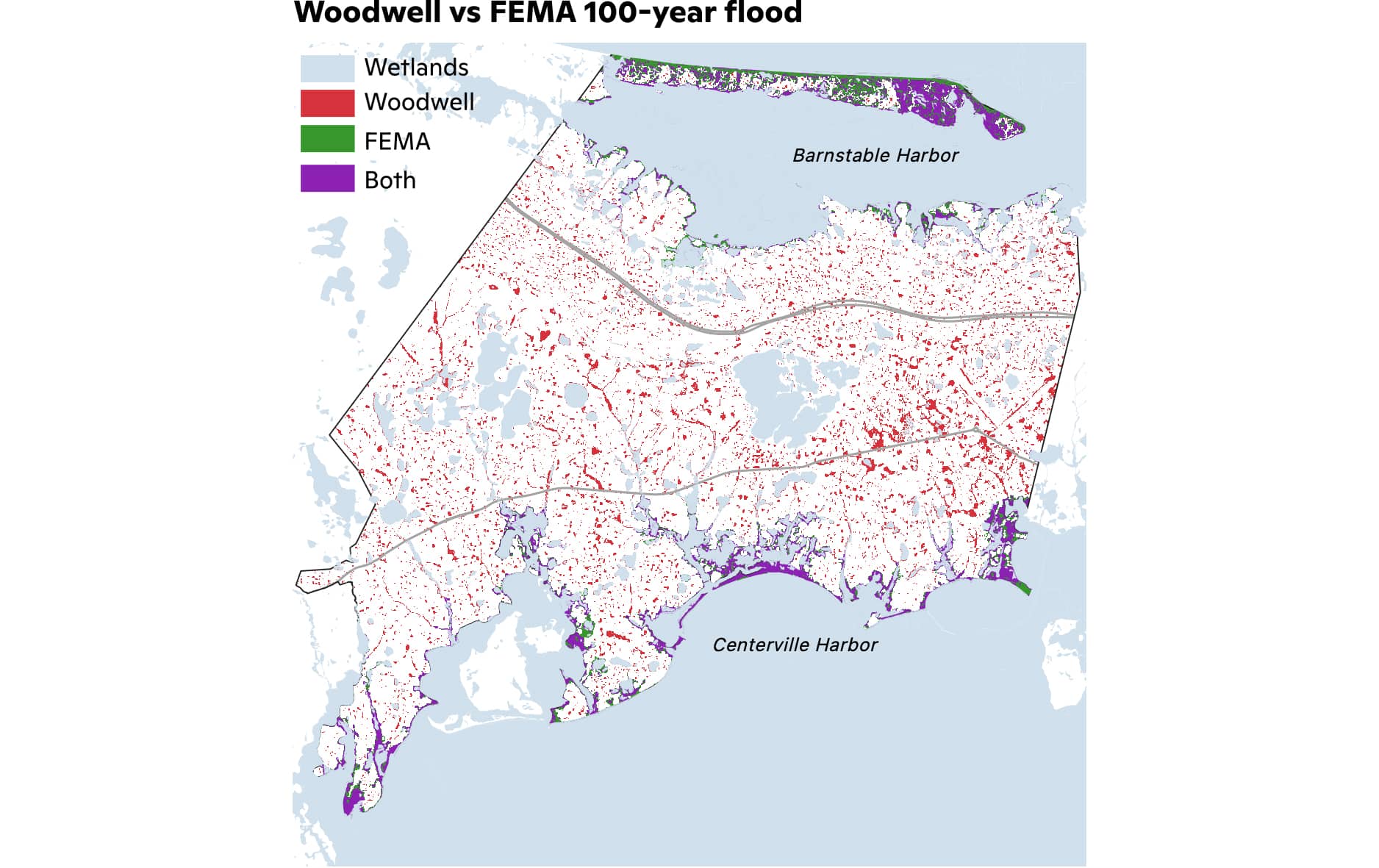
Figure 1. Woodwell vs FEMA 100-year flood. The flood extent comparison between Woodwell’s flood model results and the current FEMA flood maps for Barnstable, MA. Areas where only FEMA predicts flood risk are shown in green, areas where only Woodwell predicts flood risk are shown in red, and areas where both predict flood risk are shown in purple. The Woodwell data shows the maximum extent based on both the 100-year pluvial/riverine and the 100-year coastal floods.
Present and future flood risk
The primary flood risk in Barnstable, MA is coastal flooding. In Figure 2, we show the extent of the 100-year flood from both storm surge and rainfall for Barnstable. The highest depth values of around 5.9 ft (1.8 m) occur in the Sandy Neck Dunes with several trails underwater, as well as Sandy Neck Colony. Several coastal areas such as Hyannis south to Veterans Memorial Park, as well as Long Beach Road along southern Barnstable all approach at least 5.25 ft (1.6 m) of flood inundation. Along the intersection of Long Beach Road and Craigville Beach Road inundation depths exceed 6.5 feet (2 meters). Inland flooding occurs in some locations. An example is along Massachusetts Route 28 outside of the airport and continuing west alongside roads and parking lots next to Iyannough Road. We mask wetland areas to focus the analysis on locations where human life and property are at risk.
Approximately 80% (692 out of 874) of the coastal defenses (specifically revetments) in Barnstable are overtopped in the current simulation of a 100-year storm surge. A revetment is considered overtopped if any section of the revetment has a flood depth greater than zero ft associated with it. To mitigate future flood risk, this is a critical aspect the city can further explore to better protect lives and property along the coast. Areas around Centerville Harbor are among the most vulnerable to coastal flooding, making them a top priority, along with regions near Hyannis Harbor.
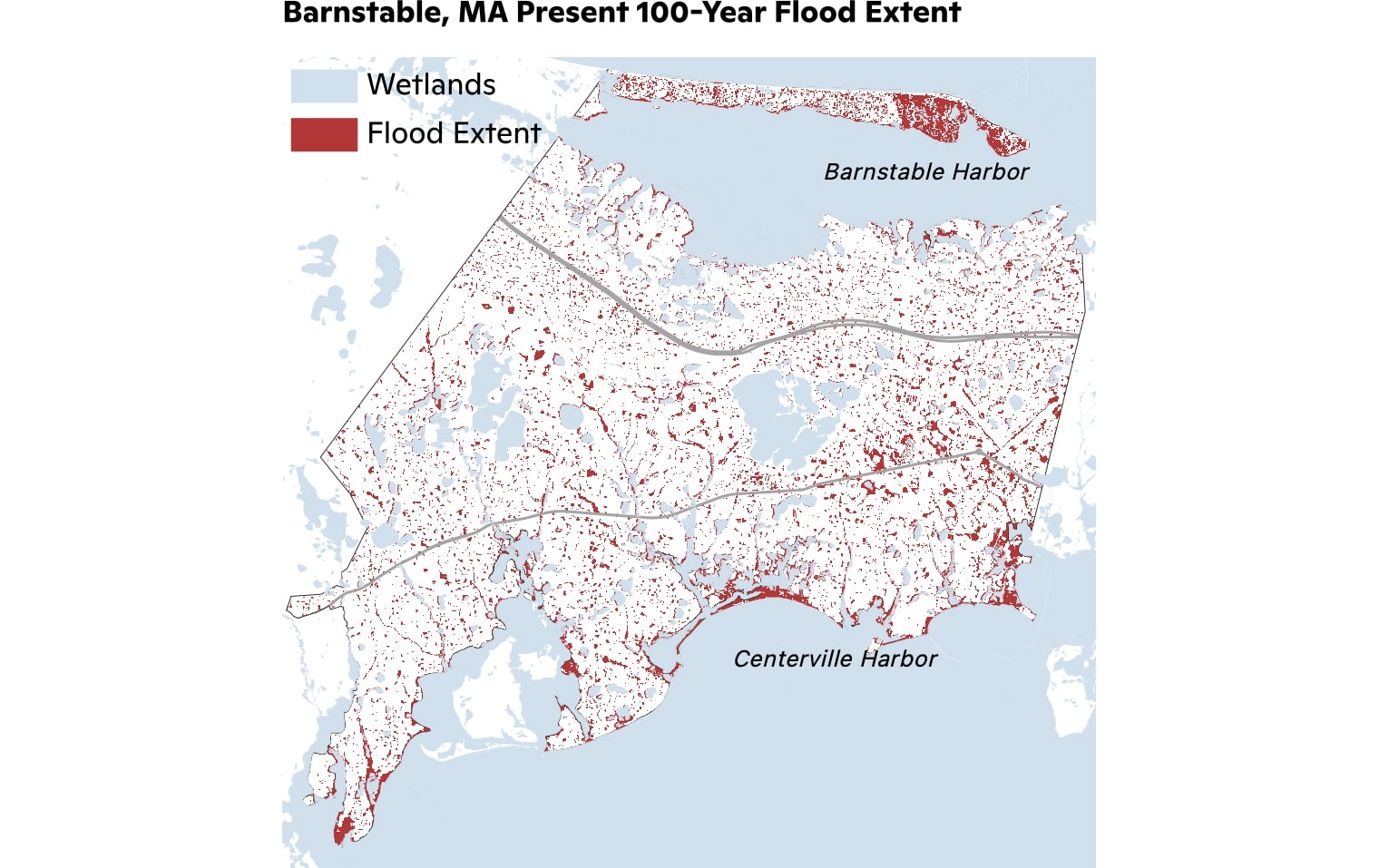
Figure 2. Present-day 100-year flood. The flood extent, quantified as having a depth of at least 0.5 ft (0.15 m), for Barnstable, MA. The maximum extent from the 100-year pluvial/riverine and 100-year coastal flood is shown.
Future flood risk is most notably seen along coastal areas consistent with present flood risk and some increased risk as shown in Figure 3. The largest changes in extent are highlighted on Figure 3 such as Sandy Neck Dunes and the coastal areas of Hyannis and Osterville. Areas between the intersection of Massachusetts Route 28 outside of the airport and Iyannough Road going west also see increased flood extents. This change in pluvial flood risk is due to projected increases in rainfall from present between 1.14 inches to 1.77 inches (29–45 mm), as shown in Table 1, the coastal flood extent is impacted by an increase of sea level (1.31 feet; 0.4 meters by 2050 and 2.07 feet; 0.63 meters by 2070). We also present several flood risk metrics in Table 2. Presently, just over 15% of the structures in Barnstable are vulnerable to the 100-year flood. That number increases to around 16% by midcentury and then to almost 18% by late century. The average flood depth in Barnstable increases by 0.56 ft (0.17 m) through the 21st century, while the area flooded increases from almost 11% in the present day to over 14% by late century.
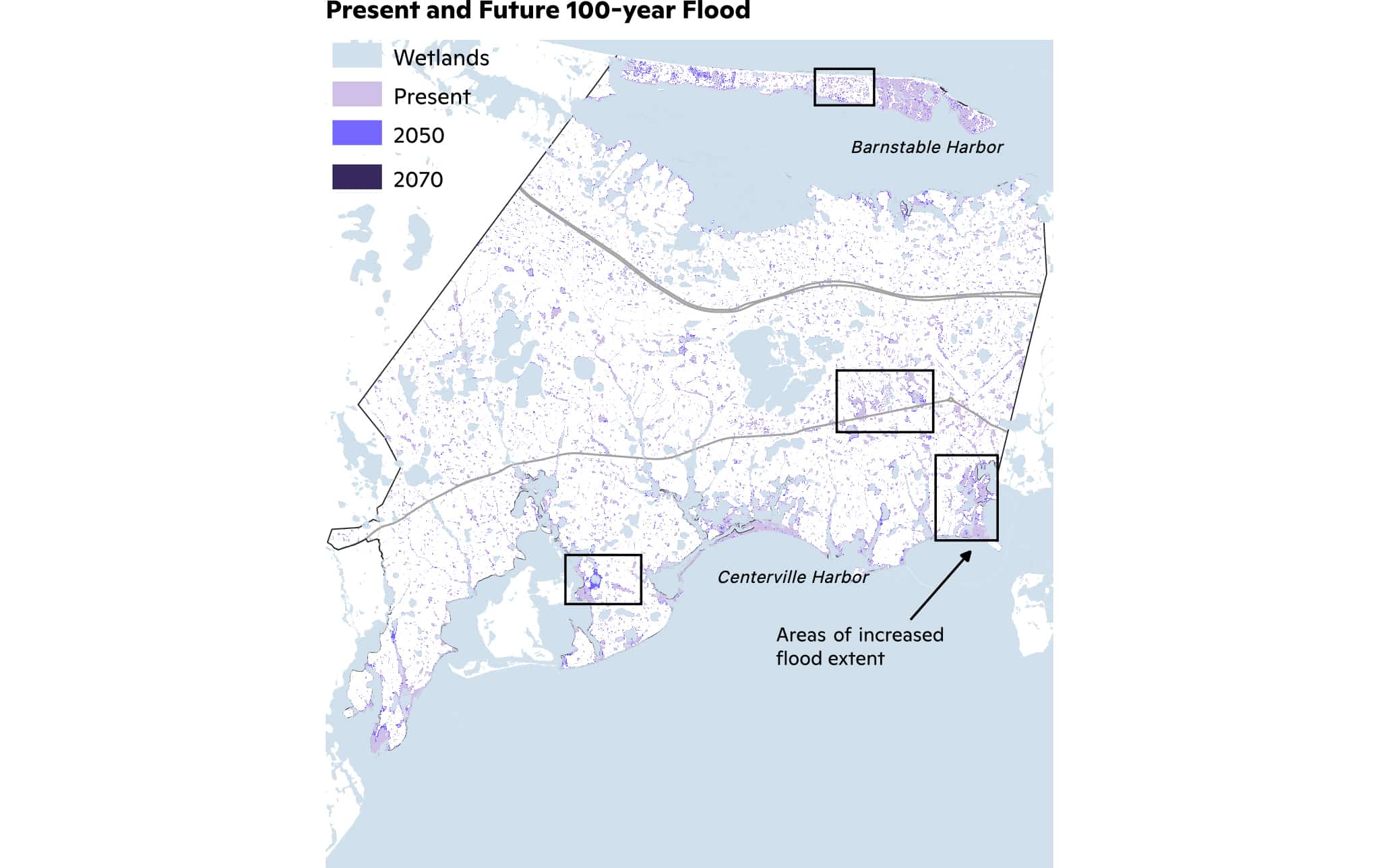
Figure 3. Present-day and future 100-year flood. The flood extent, quantified as having a depth of at least 0.5 ft (0.15 m), for Barnstable, MA. The maximum extent for the 100-year pluvial/riverine and 100-year coastal flood is shown. Areas with increased future extent are boxed.
Precipitation depths were evaluated for multiple return periods (2, 5, 10, 25, 50, 100, and 500 years) and time durations (1, 2, 3, 6, 12, and 24 hours). Figure 5 depicts the QS and NS future-estimated 24-hour rainfall depths for each return period for Tucson International Airport, compared to NA14. Results reveal very similar 24-hour rainfall totals between both scenarios (SSP2 and SSP5) for all return periods among each respective approach (i.e., QS and NS), suggesting that the differences in climate change assumptions between the two scenarios ultimately have little influence on extreme precipitation for this location and future time period. Additionally, the annual maxima of the climate models for this region could contain more noise (interannual variability) than signal (climate warming) resulting in very similar results between scenarios. Moreover, available moisture within EPC’s relatively dry climate may be an additional factor in the similarity between the SSP scenarios. The future-projected NS rainfall depths track closely with the NA14 upper 90% CI for SSP2 and SSP5. While NS results are lower than the QS results, they still represent an increase in future extreme precipitation, signifying that the current NA14 upper 90% confidence bound rainfall for each return period could become the new mean rainfall for each return period.
Present | 2040–2060 | 2060–2080 | |
Area flooded | 10.85% | 13.14% | 14.48% |
Average depth | 2.56 ft (0.78 m) | 2.92 ft (0.89 m) | 3.12 ft (0.95 m) |
Structures flooded | 3,664 (15.13%) | 4,083 (16.87%) | 4,341 (17.93%) |
Table 2: Flood risk metrics for mid- and late-21st century in Barnstable. The percent of land area (excluding wetlands) flooded and the number of buildings (and percent of total structures) flooded for Barnstable of the present-day, 2040–2060, and 2060–2080 100-year rainfall and storm surge events.
Stormwater system vulnerability
In addition to flood extents, an analysis of the flood model results for the Barnstable stormwater system was conducted to identify bottlenecks in the system. Any manholes or catch basins (sometimes referred to as drainage basins) that overflowed during the simulation were considered flooded. Conduits (pipes) that are capacity-limited (also referred to as at-capacity) were also identified. Capacity-limited is defined as when flow entering the pipe is greater than what the conduit can convey. We show capacity-limited pipes to identify any pipes that may be undersized or undersloped. These pipes may be responsible for causing flooding or upstream backwater conditions to occur at manholes or catch basins. Such pipes would be good starting points when investigating where to perform stormwater system upgrades.
Barnstable’s stormwater system shows high spatial distribution in the hot spots of vulnerability to the 100-year rainfall event. In Table 3, we show the number and percentage of manholes and catch basins flooded and capacity-limited conduits for the present-day, 2040–2060, and 2060–2080 100-year events. In Figure 4, we show the locations of concentrations of manholes and catch basins flooded as a heat map, as well as which conduits are capacity-limited. During the present-day 100-year rainfall event, 90% of all conduits in Barnstable’s stormwater system are capacity-limited. From the mid-21st century to the late-21st century, the number of capacity-limited conduits increases minimally by 1%. The percentage of manholes and catch basins flooded is significantly smaller compared to the conduits but a similar narrow upward trend is expected with 29.2% of the catch basins and 52.3% of the manholes flooded by the late-21st century. The difference in proportion of manholes/catch basins flooded and conduits at-capacity indicates that the stormwater system is able to reduce street flooding even when the stormwater pipes are filled. We identified several hot spots of stormwater flooding throughout Barnstable. These include the village of Hyannis in southeast Barnstable northward toward the Cape Cod Gateway Airport and the village of Barnstable along Barnstable Harbor.
It is important to note that we show all conduits that are capacity-limited regardless of the duration they were capacity-limited during the simulation. The vast majority of conduits in the present time period that were at-capacity (81%) spent only 36 seconds in a limited flow state. We include these conduits in the flooding metrics to give a system-wide perspective on the capacity of the stormwater system.
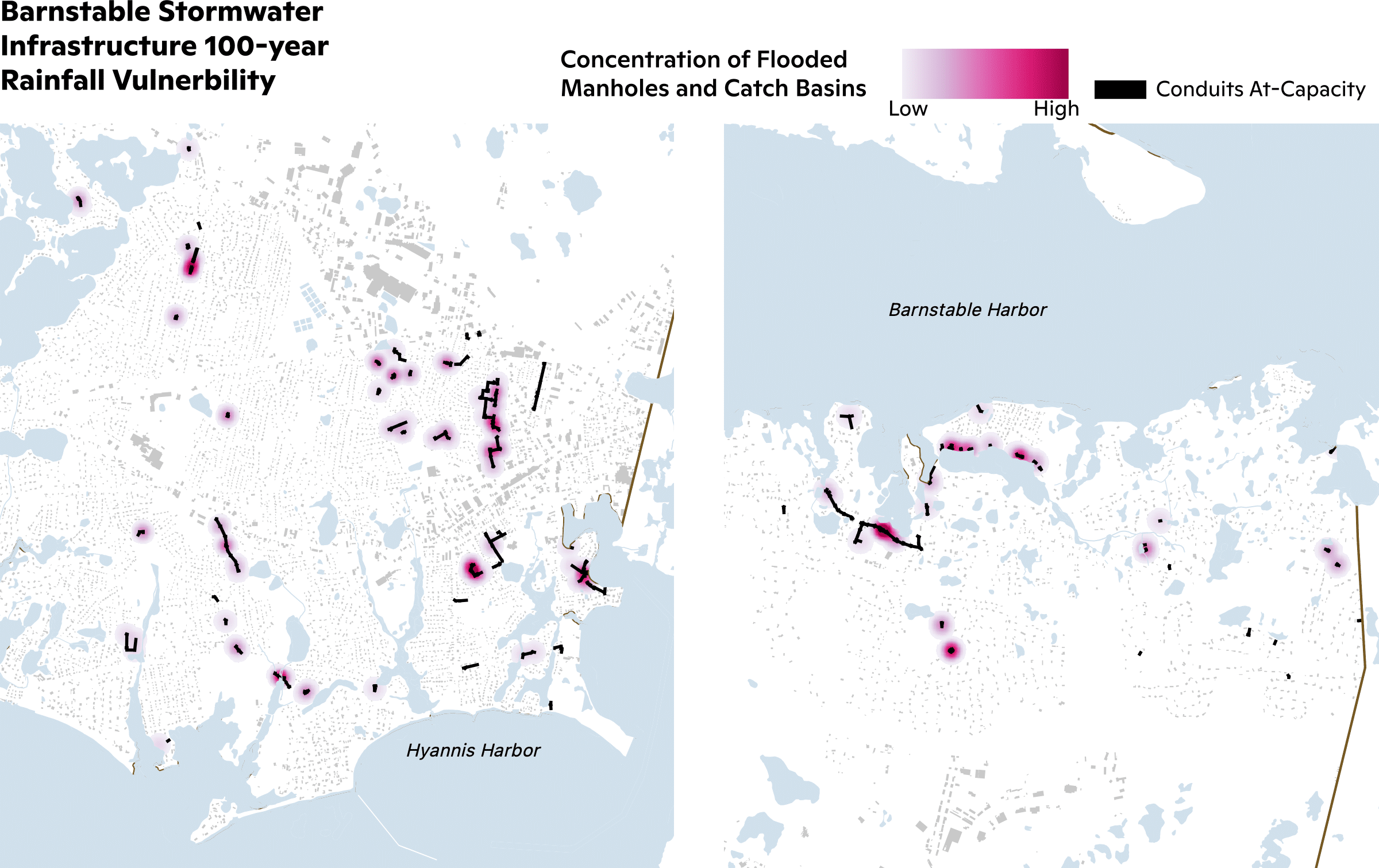
Present | 2040–2060 | 2060–2080 | |
Manholes | 199 (50%) | 206 (51.8%) | 208 (52.3%) |
Catch basins | 193 (27.1%) | 202 (28.3%) | 208 (29.2%) |
Conduits | 961 (90.6%) | 967 (91.1%) | 972 (91.6%) |
Figure 4: Barnstable stormwater system flooding heat map. The concentration of flooded manholes and catch basins shown as a heat map for the present-day 100-year rainfall event. Areas with no flooded manholes or catch basins are shown in white. Capacity-limited conduits are shown in black.
Table 3: Barnstable stormwater system flooding. The number, and percentage of total, flooded manholes and catch basins and capacity-limited conduits for the present-day, 2040–2060, and 2060–2080 100-year rainfall event.
Existing and future pump stations vulnerability
The city of Barnstable has 34 existing pump stations spread throughout the city. An additional analysis was performed where a pump station was considered flooded if 0.5 ft (0.15 m) of water was over the pump station location. This threshold is an average curb height and any flooding above this threshold would likely result in flood damages. Pump locations that have the potential to flood in the near term or by late century are another good starting point when looking at the best place to upgrade the system or elevate critical infrastructure. When looking at the present, 14 (41.2%) of the pump stations are flooded, which further increased to 16 (47.1%) in both 2050 and 2070, as shown in Table 4. Future pump station locations have been examined as well. There are a total of 16 potential future locations. Only two of these locations are at risk in the present time period with only one additional pump station at risk during the two future time periods (2050 and 2070).
Present | 2040–2060 | 2060–2080 | |
Existing | 14 (41.2%) | 16 (47.1%) | 16 (47.1%) |
Future | 2 (12.5%) | 3 (18.8%) | 3 (18.8%) |
Table 4: Barnstable existing and future pump stations. The number, and percentage of total, flooded existing and future pump stations for the present-day, 2040–2060, and 2060–2080 100-year rainfall and storm surge event.
Low lying roads vulnerability
Using the flood model output, roads across Barnstable were examined for risk of flooding. The Low Lying Roads Project from the Cape Cod Commission created a risk category for some of the roads in Barnstable, however this project focused on storm surge-induced flooding and not extreme rainfall events, such as the 1-in-100 year rainfall event, modeled here. This analysis highlights the same areas that the Low Lying Roads Project found to be at risk, as well as large areas inland, a direct result of this work including rainfall events and not solely looking at storm surge. Figure 5 shows a zoomed-in look of several southern villages (Hyannis, Centerville, Osterville, and Marstons Mills) in the town of Barnstable as this area is where a large number of the flooded roads occur. In this analysis, a road is considered flooded if the flood depth exceeds 0.5 ft (0.15 m) along any portion of the road line segment because exceeding this curb height threshold would likely result in flood damages. For context, 6 inches of water can reach the bottom of most passenger vehicles and cause engine damage.
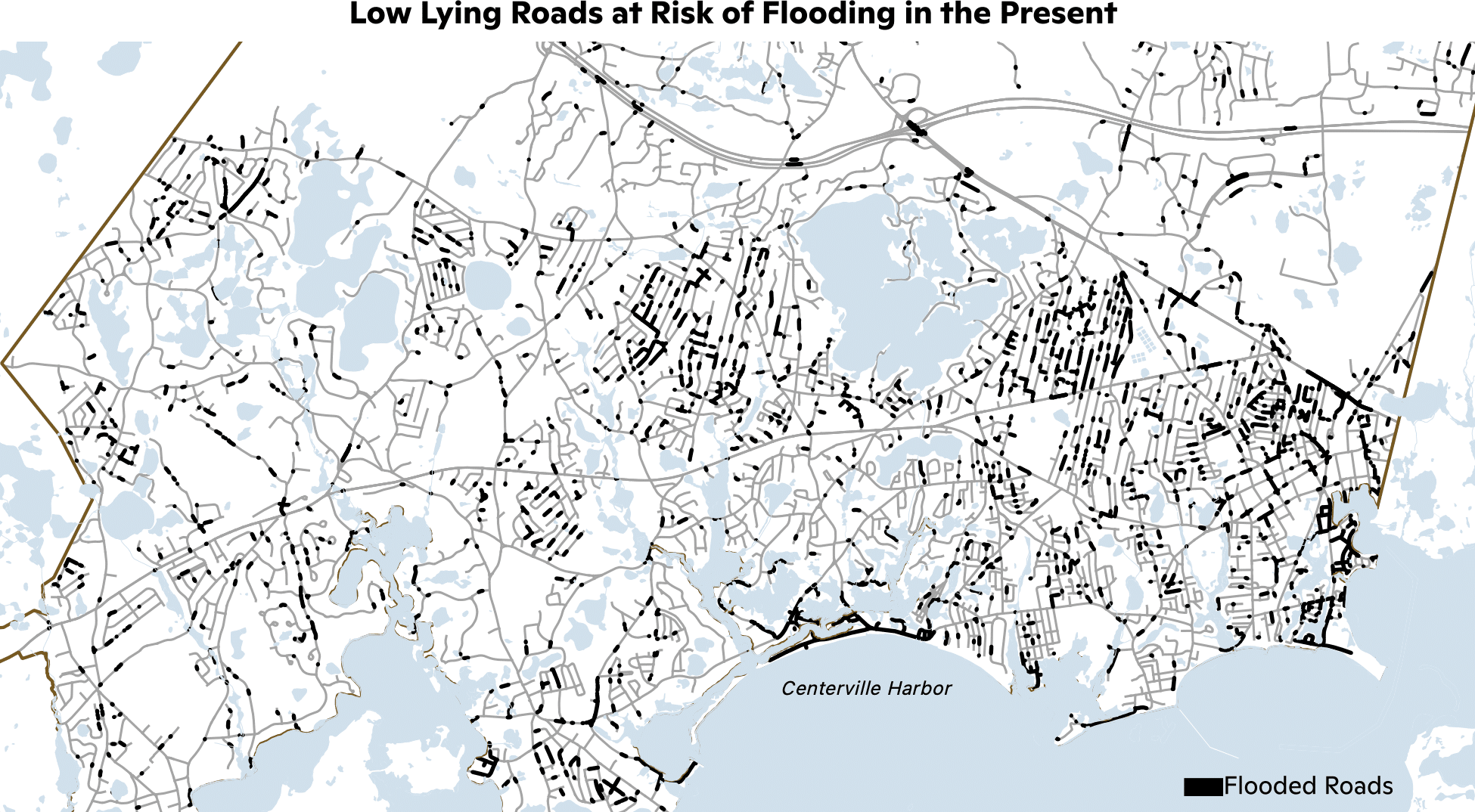
Figure 5: Barnstable low lying roads at risk. The majority of roads at risk are in southern Barnstable highlighted here. The largest concentration of roads at risk in the present-day 100-year rainfall and storm surge event is in the village of Hyannis. The low lying roads that are at risk are shown in black.
Tropical cyclone storm surge risk
Our flood risk analysis includes storm surge due to sea level rise. The present-day flood run (Figure 2) includes storm surge from FEMA which includes all storm types causing surge along with tides. This does not specifically address future tropical cyclones. Here we supplement our flood risk analysis with a separate storm surge component for two time periods: present (1980–2017) and future (2021–2050). This is done using the Synthetic Tropical cyclOne geneRation Model (STORM) to create thousands of synthetic tropical storm tracks (see methodology section). The north shore and south shore were examined independently as the tides and surge vary quite substantially (i.e., over 70% of total water increase for the north shore is tidal; over 80% of south shore is storm surge). For the north shore, the max value from surge and mean high tide is 6.56 ft (2 m; mostly tides). The south shore is more heavily impacted by surge instead of tide since it is south facing and open to approaching tropical cyclones leading to about 5.68 ft (1.73 m) of total water increase with about 4.76 ft (1.45 m) of that from storm surge alone. For the future time period, the north shore is 7.15 ft (2.18 m) with 4.46 ft (1.36 m) of that coming from tidal. The south shore increases by over one foot with a total of 6.69 ft (2.04 m) and 5.77 ft (1.76 m) from the surge. This indicates that in the coming decades the south shore of Barnstable is at increased risk of coastal flooding as a result of storm surge from approaching tropical cyclones. If we further add sea level rise, an additional 1.31 ft (0.4 m) of inundation due to tides and storm surge will occur in the future period.
Present | 2021–2050 | |
North shore | 6.56 ft (2.10 ft) | 7.15 ft (2.69 ft) |
South shore | 5.68 ft (4.76 ft) | 6.69 ft (5.77 ft) |
Table 5: Barnstable storm surge changes for the north and south shores. The amount of total water increase (tide and storm surge), and amount of total that is from storm surge in parentheses, for the present day and 2021–2050. This shows that the tides account for more of the total water for the north shore and surge accounts for more along the south shore.
Conclusion
Barnstable is currently at high risk from flooding, primarily from storm surge, and this exposure will only increase under climate change. The results presented in this study were compared to FEMA’s flood maps, revealing significant discrepancies primarily due to the exclusion of pluvial flooding in FEMA’s analysis. Our findings show an expected increase in the frequency and intensity of heavy rainfall with the probability of the present-day 100-year rainfall event to be almost twice as likely by the mid-21st century and just over twice as likely by the end of the century. Barnstable’s stormwater system will also face greater stress as rainfall intensifies with over 50% of manholes and almost 30% of catch basins flooding by the late-21st century. Pump stations in Barnstable are also at risk with over 40% flooded in the present time period along with large areas of low lying roads being inundated. Lastly, Barnstable does have an increased risk of storm surge from tropical cyclones with about a 0.66 ft (0.2 m) storm surge increase for the north shore and a 0.98 ft (0.3 m) increase for the south shore. This report provides insight into the climate vulnerability of the city of Barnstable, where an increasing number of buildings and areas will be exposed to flood waters by the end of the century.
Methodology
To simulate flood risk we use a coupled version of the LISFLOOD-FP v8.1 flood model (LISFLOOD-FP developers, 2022; Shaw et al., 2021) and the Environmental Protection Agency’s (EPA) Stormwater Management Model (SWMM). LISFLOOD-FP is a two-dimensional raster hydraulic model that solves all terms of the shallow water equations. LISFLOOD-FP has been extensively used from the river reach scale to continental simulations and we refer the reader to Shaw et al. (2021) for a detailed explanation of LISFLOOD-FP. SWMM was introduced in 1971 by the USA Environmental Protection Agency and has been continuously developed since. SWMM is a one-dimensional stormwater system model solving the one-dimensional Saint-Venant equations.
LISFLOOD-FP and SWMM are coupled based on the methodology presented in Leandro and Martins (2016). SWMM and LISFLOOD-FP were coupled using SWMM’s dynamic link library (DDL). LISFLOOD-FP’s source code was modified to allow for bidirectional interaction between the two models at outfalls, catch basins, and manholes by calling SWMM functions during each LISFLOOD-FP time step. Time step synchronization between LISFLOOD-FP and SWMM is controlled by LISFLOOD-FP. Flow from LISFLOOD-FP to SWMM through manholes and catch basins are governed by the orifice and weir equations. Flood volumes that occur at manholes and catch basins are transferred to LISFLOOD-FP. Further detail on flow interactions can be found in Chen et al. (2016).
To create storm surge estimates for tropical cyclones, thousands of years of synthetically (statistically) generated tropical cyclones are expanded into 2D wind and pressure fields using the parametric model of Holland 1980. We use tracks from the STORM dataset (Bloemendaal et al., 2020) which was derived from outputs of four global climate models (GCMs) (Bloemendaal et al., 2022). The historical tropical cyclones are compared against the International Best Track Archive for Climate Stewardship (IBTrACS) during 1980-2017, and the GCM period is 2021-2050. Wind fields are then converted to wind stress at the ocean surface using a quadratic function of windspeed and a drag coefficient according to Peng et al., (2013).
The aforementioned tropical cyclones from STORM are then used in the Regional Ocean Modeling System (ROMS) to create storm surge peak values. ROMS is a free-surface, terrain-following, primitive equations ocean model primarily developed by University of California, Rutgers University, and the French National Research Institute for Sustainable Development (Shchepetkin and McWilliams 2005, Haidvogel et al., 2008). For this project, tropical cyclones passing within two degrees (~222 km) of the gridded domain of Barnstable are considered for their storm surge impact.
All flood model results show flooding above 15 cm (~0.5 ft) as this is an average curb height and any flooding above this threshold would likely result in flood damages. All areas that are wetland and permanent water cover as determined by National Wetland Inventory (https://fwsprimary.wim.usgs.gov/wetlands/apps/wetlands-mapper).
Three time periods were used for this study: 2000–2020 (also referred to as present day), 2040–2060, and 2060–2080. These time periods can also be interpreted as warming levels in the context of climate policy. The 2000–2020, 2040–2060, and 2060–2080 periods correspond to 1, 2 and 3 degrees Celsius of warming respectively. For each time period, a pluvial/riverine flooding run and a coastal flooding run were performed. We combine the two runs by taking the maximum depth for each pixel across the two model runs unless otherwise noted.
Any analysis involving structures used the USA Structures dataset (https://gis-fema.hub.arcgis.com/pages/usa-structures). This dataset was created through a collaboration between DHS, FIMA, FEMA’s Response Geospatial Office, Oak Ridge National Laboratory, and the U.S. Geological Survey.
The following are the coupled LISFLOOD-FP and SWMM inputs:
- Rainfall
- A | Historical rainfall
The 24-hour 1-in-100 year rainfall event was used from NOAA Atlas 14 (NA14) point precipitation frequency estimates for Barnstable, MA (Perica et al., 2015). The temporal distribution, also from NOAA Atlas 14, of the 24-hour rainfall is taken from the combined cases of the four quartiles and uses the 90% cumulative probability. - B | Future rainfall
CMIP6 climate model data were bilinearly interpolated to a 1-km grid and then bias-adjusted using phase 3 of the Inter-Sectoral Impact Model Intercomparison Project (ISIMIP) version 2.5 methodology (ISIMIP3BASD v2.5) (Lange, 2019; Lange, 2021). High-resolution, 1-km Daymet reanalysis data (Thornton et al., 2022) were selected as the observation dataset for bias adjustment. We utilize a nonstationary (NS) approach to estimate future-projected extreme rainfall. In the NS approach, precipitation estimates are calculated for the entire time period (i.e., 1971–2100) using a temporal parameter to represent changes in extreme precipitation through time. NOAA recommends using a NS approach since it considers the whole time series in addition to any trends in the data, offering a more robust analysis and more stable estimate of future extreme precipitation in a changing climate. The NS approach is better suited for engineering applications as future relative changes are more realistic compared to a quasistationary approach. We use a regional fitting method to estimate the parameters of the Generalized Extreme Value (GEV) distribution. For each target pixel, a 40-mile radius is used to capture the annual maxima of the surrounding pixels. Each pixel’s annual maxima is given a weight using a triweight kernel function based on distance (e.g., pixels ≥ 40 miles have zero weight). The log-likelihood function of the GEV distribution is then minimized with the Nelder-Mead algorithm using the annual maxima and pixel weights to estimate the GEV parameters. The beta distribution of penalized coefficients ranging between -0.5 and 0.5 is used to constrict the shape parameter as specified by NOAA.To estimate future daily precipitation frequency estimates (PFEs), the biases (ratio) between the baseline period and the NA14 daily PFEs are calculated and then multiplied by the future climate model daily PFEs. We assume the daily temporal distribution of rainfall does not change in the future so we continue the use of the historical temporal distribution.
- A | Historical rainfall
- Digital Elevation Model
The USGS CoNED Topobathy DEM (Compiled 2016): New England was used to create the Barnstable, MA elevation domain. The resolution of the raw data was 1m. The final DEM resolution was set to 5m to sync better with the stormwater system. - Friction coefficients
Friction coefficients, or Manning N values, were determined based on the land cover type of the area. The 2019 land cover was used for this from the National Land Cover Database (NLCD). Based on each classification of land cover, an associated friction coefficient is provided. See table here: https://rashms.com/wp-content/uploads/2021/01/Mannings-n-values-NLCD-NRCS.pdf - Infiltration
To calculate soil infiltration rates, the USDA Soil Survey Geographic Database (SSURGO) for Massachusetts was used to obtain the soil hydrologic groups. These hydrologic groups have defined infiltration rates depending on the type of soil. Infiltration values per hydrologic group were used from Musgrave (1955). These rates in combination with the NLCD impervious surface percentages were used to compute more accurate infiltration rates. The impervious surfaces take into account built-up areas where rainfall will not be able to infiltrate. We do not incorporate the impact of stormwater systems to convey runoff from streetscapes. - Tide
Tidal data was retrieved from the NOAA Tides and Currents. Since Barnstable has a north shore and a south shore values were obtained for both. For the north shore, the mean high water, 1.319m and the mean low water, -1.574m were used. For the south shore, the mean high water, 0.173m and the mean low water, -0.373m were used. These values are relative to the vertical datum NAVD88. - Storm surge
The FEMA flood insurance study reports were used to obtain 1-in-100 year storm surge values for points along the coast of Barnstable. We do not account for shifting tropical cyclone distributions as this is beyond the scope of this study, however a separate section was included discussing the potential changes in storm surge as a direct result of tropical cyclones. - Sea level rise
Sea level rise data for Barnstable, MA was taken from the IPCC AR6 Sea Level Projection Tool. We use the SSP5-8.5 Low Confidence scenario in order to maintain consistency with the future rainfall analysis. We use the low confidence scenario (which refers to greater Antarctica and Greenland ice sheet melting) because global sea level rise projections continue to increase as new data comes to light (Garner et al., 2018). Moreover, the scientific community’s understanding of Antarctica’s contribution to sea level rise is not strong enough to meaningfully differentiate between SSP5-8.5 and other scenarios except for SSP1-2.6 (van de Wal et al., 2022). However, there is only a 0.1% chance that society will experience the SSP1-2.6 scenario (Zeppetello et al., 2022). Additionally, the SSP5-8.5 Low Confidence scenario is roughly equivalent to the NOAA Intermediate scenario presented in the Interagency Sea Level Rise Scenario Tool. - Water depth startfile
Due to the DEM containing bathymetry elevations in Cape Cod Bay to the north and Nantucket Sound to the south, the model was initialized with starting water elevations representing a standard tide level. First, a permanent water mask was used to locate pixels that should be initialized with water elevations. Then, if the elevation of a pixel was less than the mean low water tide elevation, -1.574m NAVD88 for the north and -0.373m NAVD88 for the south in this case, then the starting water elevation for the pixel would be the tide level minus the DEM value. Future scenarios took sea level rise into account and adjusted the tides by the increase of sea level.
Methodology references
Bloemendaal et al. (2020). Generation of a global synthetic tropical cyclone hazard dataset using STORM. Sci Data 7(40). https://doi.org/10.1038/s41597-020-0381-2
Bloemendaal et al. (2022). A globally consistent local-scale assessment of future tropical cyclone risk. Science Advances 8(17). https://doi.org/10.1126/sciadv.abm8438
Chen, A. S., Leandro, J., & Djordjević, S. (2016). Modelling sewer discharge via displacement of manhole covers during flood events using 1D/2D SIPSON/P-DWave dual drainage simulations. Urban Water Journal 13(8), 830-840. https://doi.org/10.1080/1573062X.2015.1041991
Garner, A. J., Weiss, J. L., Parris, A., Kopp, R. E., Horton, R. M., Overpeck, J. T., & Horton, B. P. (2018). Evolution of 21st century sea level rise projections. Earth’s Future 6(11), 1603-1615. https://doi.org/10.1029/2018EF000991
Haidvogel, D. B. et al. (2008). Ocean forecasting in terrain-following coordinates: Formulation and skill assessment of the Regional Ocean Modeling System. J. Comput. Phys. 227, 3595–3624. https://doi.org/10.1016/j.jcp.2007.06.016
Hosking, J. R. (1990). L-moments: Analysis and estimation of distributions using linear combinations of order statistics. Journal of the Royal Statistical Society: Series B (Methodological) 52(1), 105-124. https://www.jstor.org/stable/2345653
Lange, S. (2019). Trend-preserving bias adjustment and statistical downscaling with ISIMIP3BASD (v1. 0). Geoscientific Model Development 12(7), 3055-3070. https://doi.org/10.5194/gmd-12-3055-2019
Lange, Stefan. (2021). ISIMIP3BASD (2.5.0). Zenodo. https://doi.org/10.5281/zenodo.4686991
Leandro, J. & Martins, R. (2016). A methodology for linking 2D overland flow models with the sewer network model SWMM 5.1 based on dynamic link libraries. Water Science and Technology 73(12), 3017-3026. https://doi.org/10.2166/wst.2016.171
LISFLOOD-FP developers. (2022). LISFLOOD-FP 8.1 hydrodynamic model (8.1). Zenodo. https://doi.org/10.5281/zenodo.6912932
Musgrave, G.W. (1955) How Much of the Rain Enters the Soil? In: The United States Department of Agriculture, Ed., Water: Year book of Agriculture, The United States Department of Agriculture, Washington DC, 151-159. https://search.nal.usda.gov/discovery/delivery/01NAL_INST:MAIN/12284995130007426
Peng et al., (2013). Adjusting the Wind Stress Drag Coefficient in Storm Surge Forecasting Using an Adjoint Technique. Journal of Atmospheric And Oceanic Technology 30. https://doi.org/10.1175/JTECH-D-12-00034.1
Perica, Sanja et al. (2015). Precipitation-Frequency Atlas of the United States. Volume 10 Version 3.0: Northern States; Connecticut, Maine, Massachusetts, New Hampshire, New York, Rhode Island, Vermont. https://doi.org/10.25923/99jt-a543
Prescott, P. and Walden, A. T. (1980). Maximum likelihood estimation of the parameters of the generalized extreme-value distribution. Biometrika 67(3), 723–724. https://doi.org/10.1093/biomet/67.3.723
Shaw, J., Kesserwani, G., Neal, J., Bates, P., & Sharifian, M. K. (2021). LISFLOOD-FP 8.0: the new discontinuous Galerkin shallow-water solver for multi-core CPUs and GPUs. Geoscientific Model Development 14(6), 3577-3602. https://doi.org/10.5194/gmd-14-3577-2021
Shchepetkin, A.F. & McWilliams, J.C. (2005). The regional oceanic modeling system (ROMS): A split-explicit, free-surface, topography-following-coordinate oceanic model. Ocean Model 9, 347–404. https://doi.org/10.1016/j.ocemod.2004.08.002
Thornton, M.M., R. Shrestha, Y. Wei, P.E. Thornton, S-C. Kao, & B.E. Wilson. (2022). Daymet: Daily Surface Weather Data on a 1-km Grid for North America, Version 4 R1. ORNL DAAC, Oak Ridge, Tennessee, USA. https://doi.org/10.3334/ORNLDAAC/2129
van de Wal, R. S., Nicholls, R. J., Behar, D., McInnes, K., Stammer, D., Lowe, J. A., … & White, K. (2022). A High-End Estimate of Sea Level Rise for Practitioners. Earth’s Future 10(11), e2022EF002751. https://doi.org/10.1029/2022EF002751
Zeppetello, L. R. V., Raftery, A. E., & Battisti, D. S. (2022). Probabilistic projections of increased heat stress driven by climate change. Communications Earth & Environment 3(183). https://doi.org/10.1038/s43247-022-00524-4